6 Weathering and Soils
Nahgeib Miller
Learning Objectives
After completing this chapter, you should be able to:
- Define weathering.
- Describe the factors that control weathering and explain how they affect a particular rock.
- Define a soil.
- Describe how soils are formed.
- Describe a typical soil profile.
- Describe soils according to the Canadian Soil Classification System.
- Define and describe mass wasting and its controlling factors.
- Classify mass movements based on the nature of the slope materials, the nature of the movement, and the speed on movement.
- Explain the development of the Niagara Escarpment.
6.1 Weathering
Weathering, one of the main processes in the rock cycle, breaks down rock into all of Earth’s sediments, clays, soils, and dissolved minerals. Weathering occurs only at the surface of Earth when rock becomes exposed to and interact with Earth’s climate. Simply put, when tectonic forces cause the uplift of rock into mountain ranges, air is forced to rise to cross the mountains. This causes the water in the air to cool and condense into rain, which in turn causes weathering of the rocks in the mountain. That is, the mountains create the very conditions for weathering to act on themselves.
Erosion and mass wasting are the processes that remove the particles of rock created by weathering and transport those particles downhill. Although water is the main agent of weathering, it is also one of the main agents of erosion (with wind and gravity being the others), and we must take care to recognize the difference between the process of weathering and that of erosion: weathering creates the particles, and erosion moves them.
Factors Controlling Weathering
The speed at which rock weathers depends on a series of independent and interacting factors as follows:
- Parent Rock Makeup The main factor controlling weathering is the makeup of the parent rock. Different minerals will weather at different rates based on their solubility and reactivity, so rocks with soluble and/or reactive minerals will weather faster than rocks with resistant minerals. Furthermore, the structure of the rock will influence the rate of weathering because cracks, bedding, and cleavage (on the mineral level) allows water to infiltrate the rock. Essentially, the cracks increase the surface area of the rock that is available for reaction. Therefore, a massive rock (with no cracks or bedding) with stable minerals will weather more slowly than the same rock with fractures like joints.
- Climate Because the presence of water is a major factor controlling weathering, weathering will proceed at a faster rate in a climate that produces more rainfall. Also, the addition of heat accelerates chemical reactions, which accelerates weathering. Therefore, rocks will break down the fastest in hot, rainy climates like that in rain forests.
- Presence of Soil Cover Intuitively, one would expect that soil would block water from reaching the rocks below, thus reducing the effect of weathering. However, soil actually acts like a wet blanket covering the rock, keeping the water in contact with the rock beneath for a lot longer than if there was no soil. This accelerates the weathering process.
- Slope of the Rock The angle of the slope of the rock will affect how long the water remains in contact to facilitate the weathering process. The steeper the slope, the faster water moves, which reduces the amount of time the water is in contact with the rock, thus reducing weathering. However, while weathering decreases on steeper slopes, erosion will now dominate and vice versa.
- Time In the short term, the nature of the parent rock and the climate are the dominant factors controlling weathering; however, in the long term, the length of exposure will be dominant. This is because the weathering of rock is defined more by what is left behind after the process (the residue), and all rock tends to have similar residue if weathered long enough.
Chemical Weathering
Chemical weathering occurs when minerals in rock come in contact with and react with the constituents of air and water. These types of reactions are as follows:
- Oxidation Oxidation is the main reaction between minerals and oxygen in air and water. This produces primarily iron oxides (such hematite) from the iron-bearing silicate minerals (that is, mafic minerals), giving soils a distinctive reddish-brown colour.
- Hydrolysis Hydrolysis is the main reaction between minerals and water breaking down silicate minerals to clays. Water is a polarized molecule with a hydrogen cation and a hydroxide anion, and we can represent our ferromagnesian silicates as CA, where C is the cation and A is the silicate anion. Then the hydrolysis reaction would be as follows: H OH– + CA = COH + HA. Clays are submicroscopic to microscopic in size (less than 0.0039 mm) and therefore lead to the disaggregation of the rock because adjacent minerals are no longer held in place by one large mineral crystal. This loosens the more stable adjacent mineral, creating a particle that can be eroded (Figure 6.1).
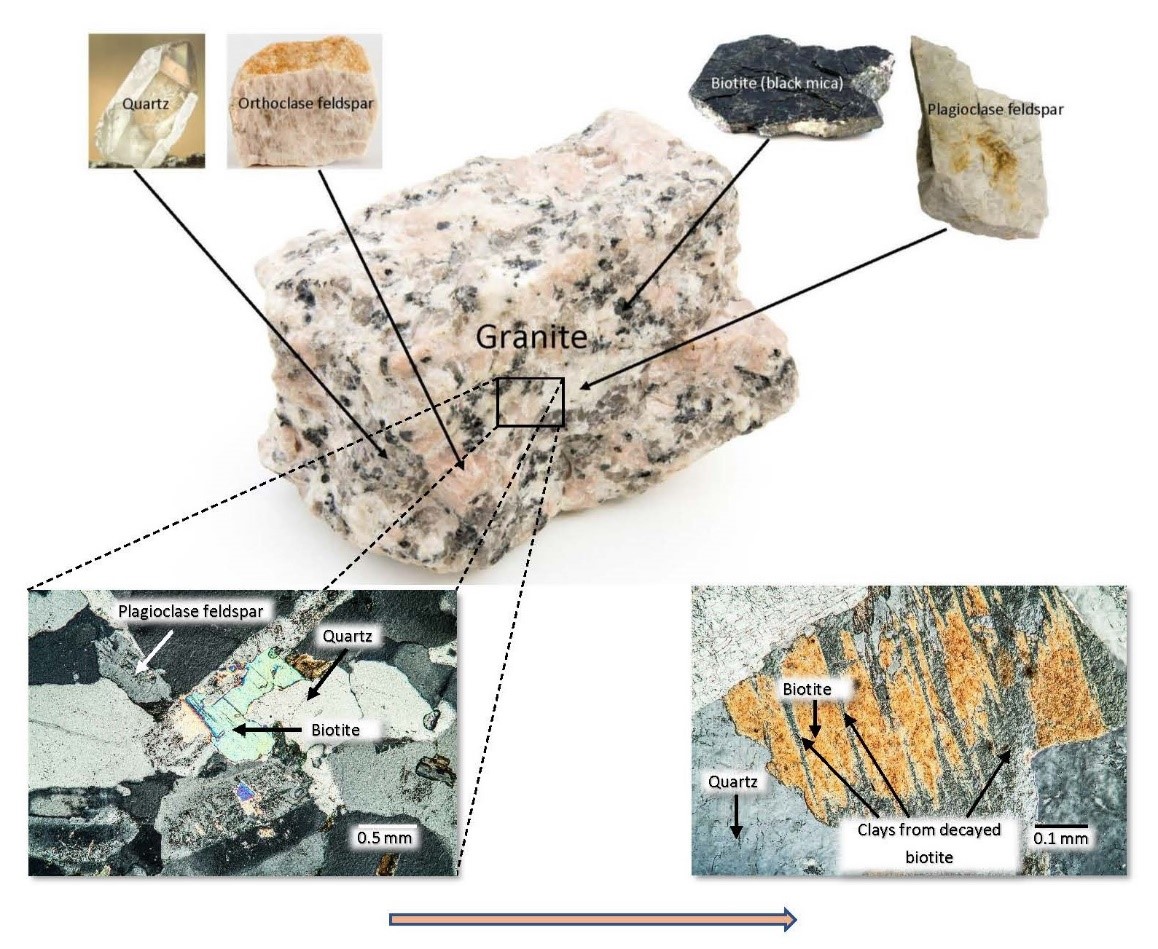
- Acid–Base Reactions As rainwater falls through the atmosphere, it reacts with carbon dioxide to create carbonic acid (H2CO3), represented chemically as H+ HCO3–. The carbonic acid reacts with silicates to produce clay, with the transformation of feldspar to kaolinite clay as an example.
- Dissolution Water is a solvent that dissolves soluble minerals on contact. The greater the solubility of the minerals, the faster the rate of weathering. Therefore, minerals that are more resistant to the foregoing processes will weather at a slower rate than minerals that are more chemically unstable. This leads to the disintegration of rock because the interlocking nature of minerals in rock is removed, releasing more stable minerals as particles (Table 6.1). In general, the most stable minerals are the ones that form last, during the cooling of molten rock (these are the minerals with more silicate-to-silicate bonds) and the least stable are the ones that form first (these are the ones with more cation–silicate bonds)!
Table 6.1. Relative stabilities of common rock-forming minerals
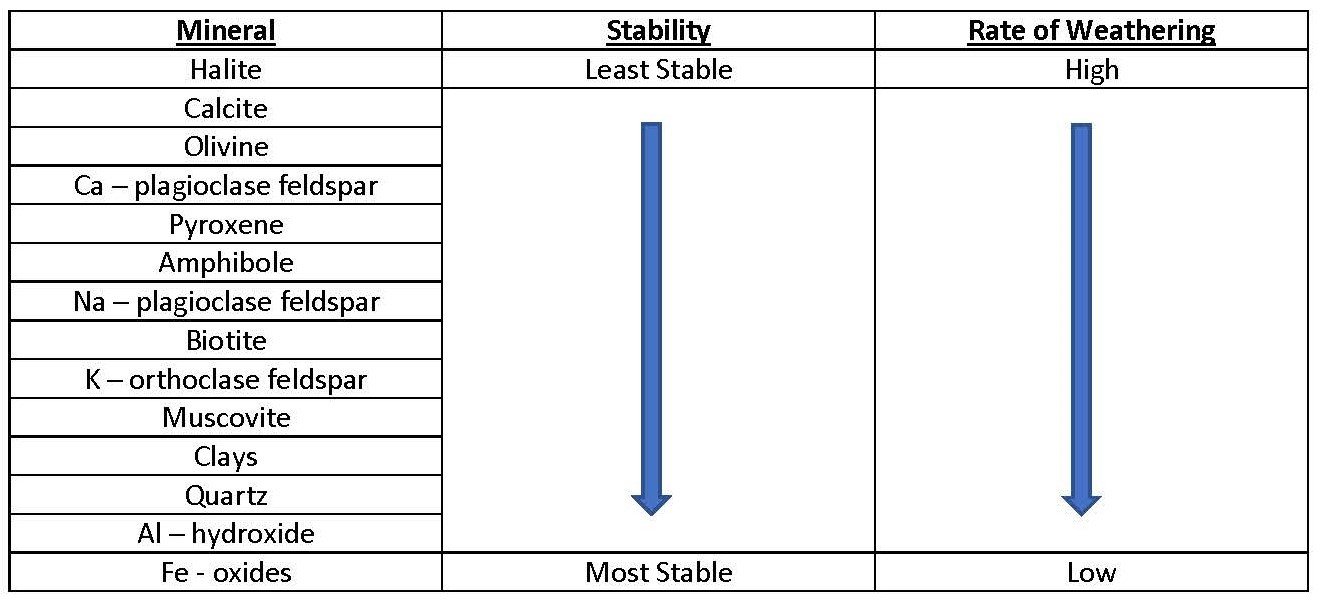
Physical Weathering
Physical weathering occurs when solid rock is fragmented by mechanical processes that do not change its chemical composition. We can see the workings of physical weathering most clearly in arid (dry) regions, where chemical weathering is minimal. Physical weathering is driven by fragmentation, which opens channels through which water and air can penetrate and react with minerals inside a rock (because of the increase in surface area). However, physical weathering does not always depend on chemical processes (chemical weathering). Some processes, such as the freezing and thawing of water in cracks, physically break up rock into particles. Fragmentation in rock is driven by the following processes:
- Natural Weakness Sedimentary and deformation structures in rock like bedding, joints, and faults create natural zones of weakness that preferentially break when subjected to physical force. Therefore, a more massive rock is more resistant to fragmentation than a similar rock that is jointed (Figure 6.2).
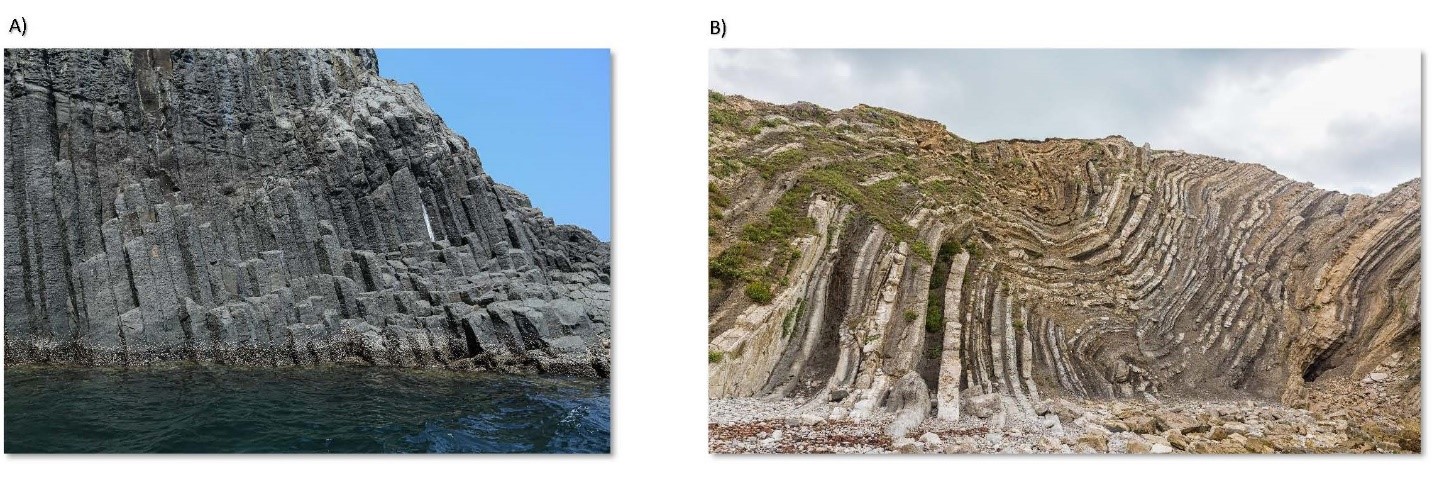
(a) Columnar joints in volcanic rock. (b) Folded beds of sedimentary rock
- Frost Wedging Natural zones of weakness allow water and air to penetrate deep into the rock. When water moving along these zones freezes, it expands, creating a larger crack, which in turn allows more water to penetrate. This process is called frost wedging. This positively reinforced cycle causes cracks (joints, bedding, faults) to continuously become larger until large pieces break off (into large particles). If these large pieces in turn have natural zones of weakness, then the process will continue break the rock apart until only small particles remain (Figure 6.3).
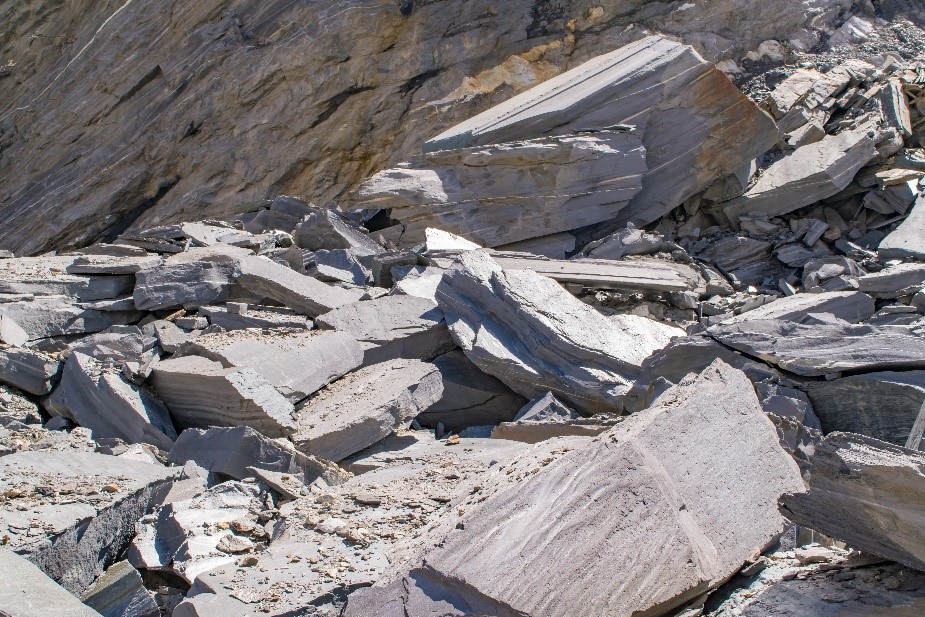
- Exfoliation Matter (apart from water) tends to expand when heated and contract when cooled. This is because the molecules that make up the matter become excited when heated and require more space to accommodate their vibrations and less space when cooled. Because heating is not uniformly distributed throughout rock, shear planes develop where expansion and contraction are more rapid. Heating and cooling start at the surface and work inward, causing the surface to expand and contract faster than rock immediately beneath, creating thin strips of rock separated by a zone of weakness in a process called exfoliation. As the strips of rock at the surface fall off, creating particles, the rock beneath is exposed and the process repeats.
- Activity of Flora and Fauna In their efforts to survive, organisms can break rock apart. For example, the roots of plants that form on rock will penetrate through natural zones of weakness in the rock because this is where water would be present. As the plants grow, the zones of weakness will open wider until pieces of rock break off. Also, burrowing animals can cause pieces of rock to break off as the animals move through the substrate, creating zones of weakness that make the rock more susceptible to frost wedging and to the activity of plant roots.
The processes of fragmentation are governed by factors that control the rate and intensity of physical weathering. These factors include:
- Parent Rock Makeup Hardness of the minerals in the rock is the main control on its susceptibility to physical weathering. The harder the constituent minerals, the less physical weathering will occur; that is, rocks with more quartz will physically weather less than rocks with more feldspars.
- Climate Higher amounts of rainfall will lead to more fragmentation; however, higher temperatures will cause chemical weathering to dominate instead of physical weathering, which dominates in colder temperatures.
- Time Given enough time, mountains of rock will be fragmented into small particles (small sediments) as the processes of fragmentation proceed at either a slow or a fast rate.
Chemical and physical weathering reinforce each other. Chemical decay and physical breakup join with rainfall, wind, ice, and snow to wear away mountains of rock. As erosion moves weathered material, it exposes fresh, unaltered rock to weathering. Chemical weathering rates are low at high altitudes where temperatures are generally low, soil is thin or absent, and vegetation is sparse. Physical weathering is greater at high altitudes and in glacial terrains, where ice tears apart the rock.
6.2 Soils
Soils are the leftover residue of weathering and are defined by what is taken out and what goes in. Rock is broken down at the surface of Earth by weathering, creating particles and transforming existing minerals into new, more stable minerals (oxides and hydroxides). The least stable minerals are removed — transported by water (if they are soluble) and/or transformed into more stable oxidized forms (for example, iron oxides) or hydroxide forms (for example, clays).
The formation of soil also depends on material that makes its way into the weathered mass. Soluble material can precipitate and become deposited in the soil as pods, lenses, and coatings, while organic material can be incorporated, usually in the uppermost sections of the soil mass.
The processes that result in the change of materials from one form to another are termed transformations, and the processes that result in the movement of materials from one zone to another within the soil are termed translocations.
Soils vary in colour, from iron-rich soils that give off bright reds and browns to black soils rich in organic matter. Soils also vary in texture; some are full of coarse material like pebbles and sand and others are composed entirely of fine material like clay. Soils are an amalgam of loose heterogenous material that is easily eroded and therefore soils do not form on very steep slopes or where altitude or frigid climate prevents plant growth.
The texture of soil determines its porosity and structure, which in turn influence how water moves through it and the vertical location of the horizons. Horizons in soil are defined by the contents of the horizons rather than by the location of layers or beds.
Air and water normally fill the pores with soil air, which is generally saturated with water vapour. Soil air can be several times richer in CO2 than atmospheric air, with half the oxygen content.
Basic soil classification is based on its texture, which is the relative composition of sand, silt, and clay. This is used to produce an engineering classification that is useful in predicting engineering characteristics of porosity, density, and water content because soils with similar texture will behave in the same way (in an engineering context) irrespective of content of the soil (Figure 6.4).
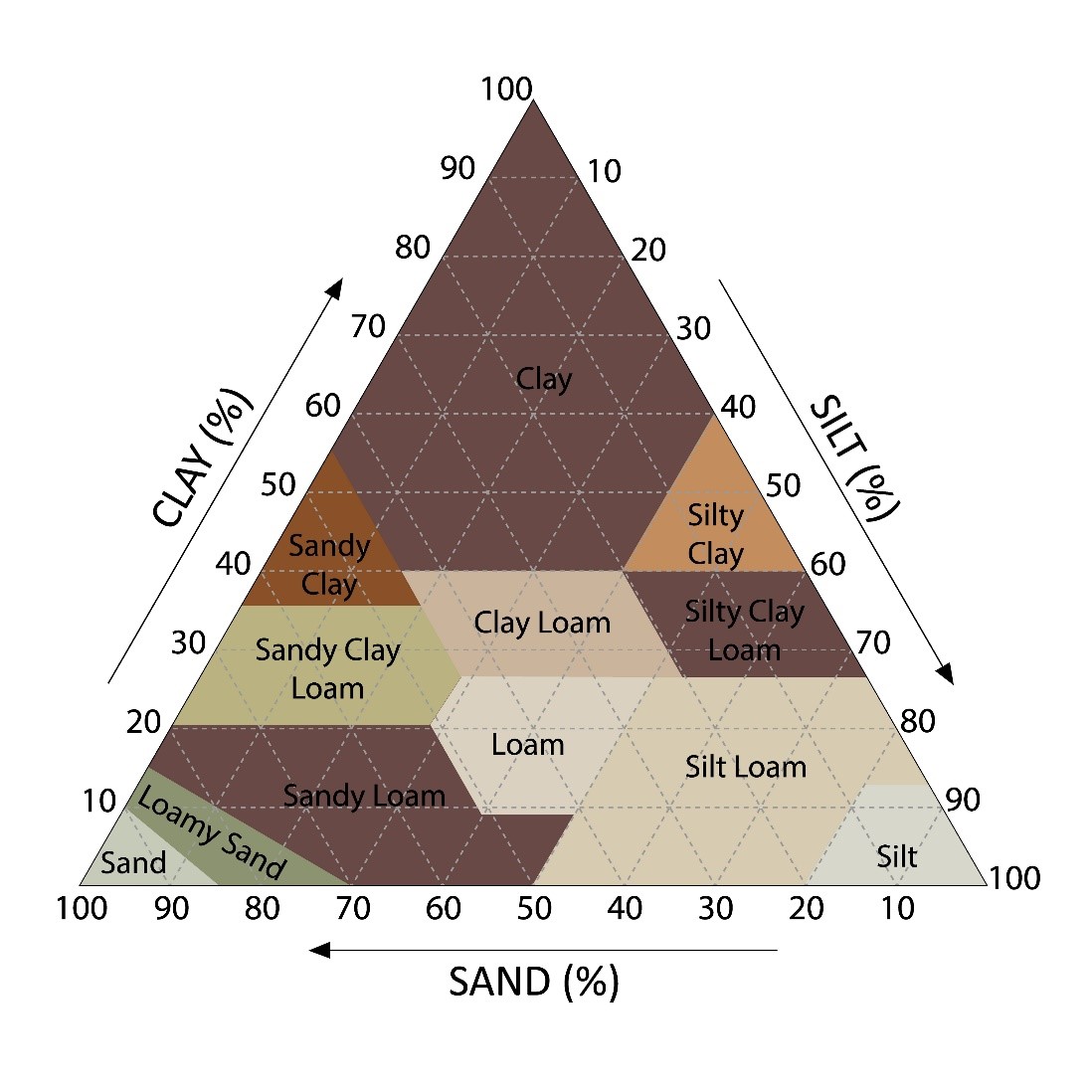
The Canadian System of Soil Classification
A more holistic classification of soil is promulgated by the Canadian System of Soil Classification and is based on the horizons present in the soil. The horizons that are present in soil are defined by the chemical and biological constituents that are indicative of and correspond with a particular horizon (Figure 6.5).
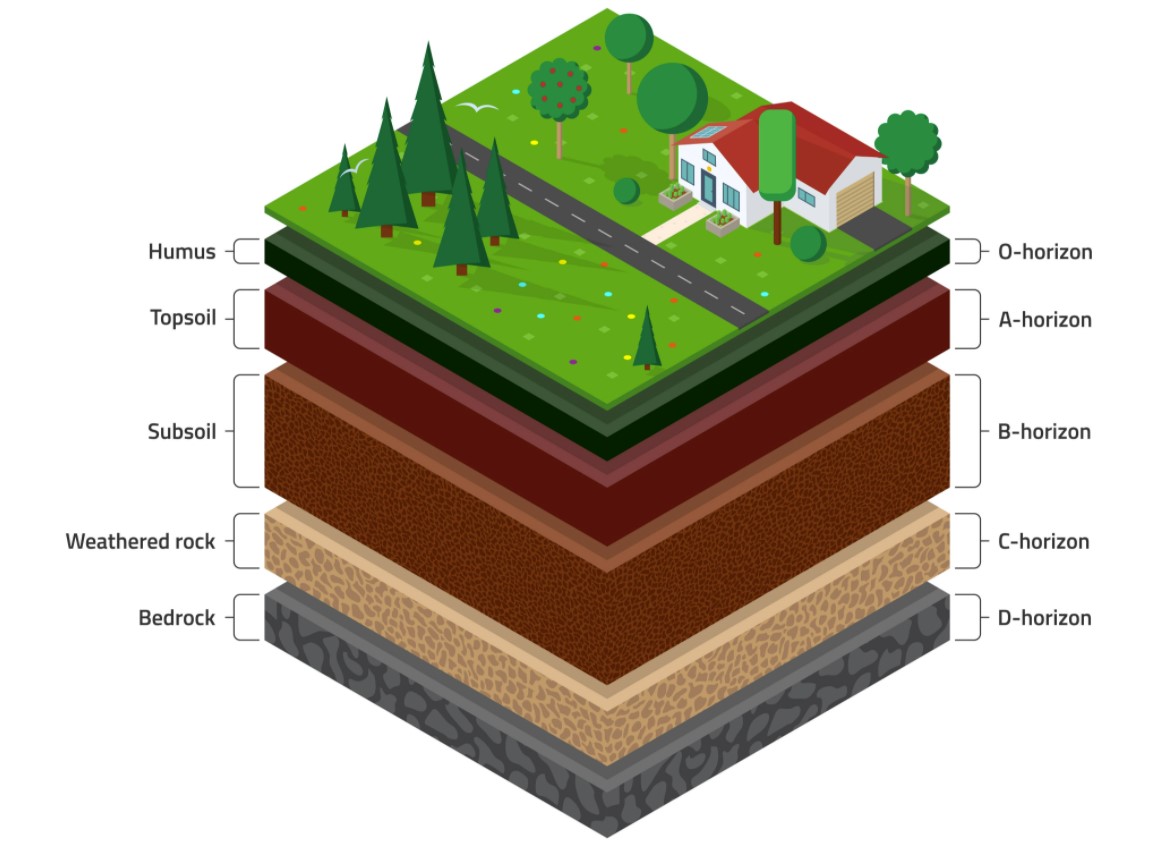
Horizons are identified from a soil profile, which is like looking at a “layer cake” slice through the soil. Be careful not to confuse horizons with layers in the soil. A soil profile may have several layers if it is transported and redeposited after creation, but the horizons may not correspond to these layers. Furthermore, the change between horizons is gradual and not as “sharp” as that in layers or bedding. As a means of deeper understanding, let us take a closer look at these horizons.
- Horizon A Horizon A forms the uppermost zone in soil and is thick in well-developed soils and thin in young soils. In well-developed soils, all of the soluble minerals would have been leached away and transported downward in the soil profile. Furthermore, the other, less stable but insoluble minerals would be transformed into clays. Therefore, the A horizon consists of an organic rich layer called the humus layer (O-horizon), with quartz and clay minerals.
- Horizon B Horizon B forms the middle of the soil profile and is the zone where the soluble minerals, leached from the A horizon, are oxidized and subsequently deposited. All other soluble minerals are leached away to the groundwater; however, soluble carbonates can be drawn up from the water table through capillary action and deposited. Capillary action occurs when interconnected pore spaces form narrow tubes along which water is drawn up due to surface tension. Therefore, the B horizon has sparse organic content and can consist of masses, nodules, lenses, and coatings of iron and aluminum oxides and of calcium/magnesium carbonates.
- Horizon C Horizon C is the zone dominated by bedrock altered by weathering. The constituents here would resemble rock more than soil, although most of the less stable minerals would have been removed or transformed into clays.
- Horizon D Horizon D is the zone dominated by unaltered/unweathered bedrock.
Ontario has a temperate climate with moderate to high rainfall and temperature. The characteristics of soils in regions of moderate rainfall and temperatures depend on climate, type of bedrock or parent rock, and the length of time the soils have to develop and thicken. Soil developed after a relatively short time on granite bedrock in a climate with moderate temperatures and humidity may differ greatly from soil formed on limestone under the same conditions. This is because the influence of the parent rock is strong. However, after many thousands of years, the differences between the two soils may dissipate or even disappear.
Both soils may develop the same clay minerals depending on the climate, and both will have lost all soluble minerals in the upper layers. Therefore, young soils are affected by the composition of the parent rock but older soils are affected mainly by climate.
Soils are also described as either residual or transported. Residual soils evolve in one place from bedrock to well-developed soil horizons. Most soils are residual and form faster where weathering is intense. Even under intense weathering, the A horizon may take thousands of years to develop to the point where it can support crops.
Transported soils may accumulate in limited areas of lowlands after they have been eroded from surrounding slopes and carried downhill. The soils in southern Ontario can be considered to be transported and can be distinguished from ordinary sediments as they are more similar in texture and composition to soils than they are to ordinary sediments, although they owe their thickness to deposition rather than to weathering in place. However, they can be confused with sediments laid down by rivers (in fluvial processes), wind (in aeolian processes), and ice (in glacial processes).
Soils form relatively slow because the most active chemical weathering occurs only during the short intervals of rain. During dry periods, reactions (chemical weathering) continue very slowly and only while moisture remains in the soil. When the soil dries out completely between rains, chemical weathering stops completely.
Based on the nature and occurrence of the horizons, soils can be classified into five hierarchical categories ranging from general to specific as follows:
- Order
- Great Group
- Subgroup
- Family
- Series
We will focus on the ten orders:
- Brunisolic soils Brunisolic soils occur in poorly to well-drained sites in a wide range of climates and floral environments. These soils are residual with a brownish B horizon and much less leaching, yielding less evidence of translocations (Figure 6.6).
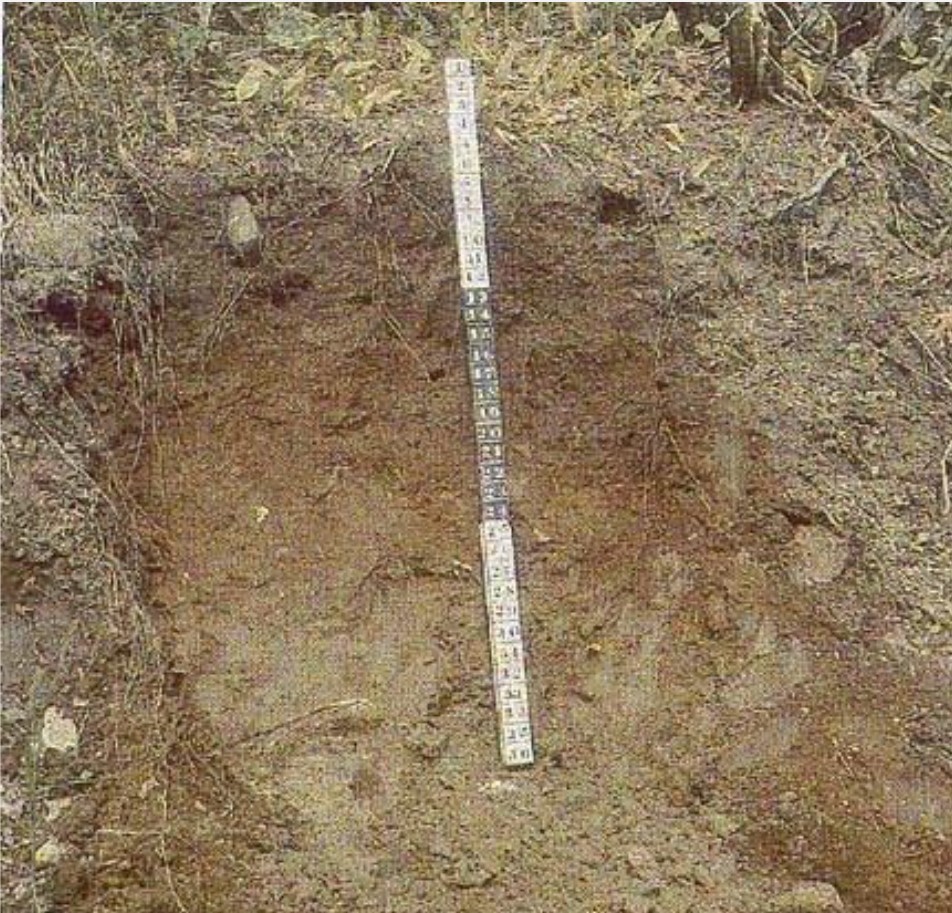
- Chernozemic soils Chernozemic soils are poorly to well-drained soils with a darkened A horizon due to organic accumulation (Figure 6.7). This is representative of grassland or grassland-and-forest environments. The B and C horizons have a high concentration of carbonates and usually form from limestones.
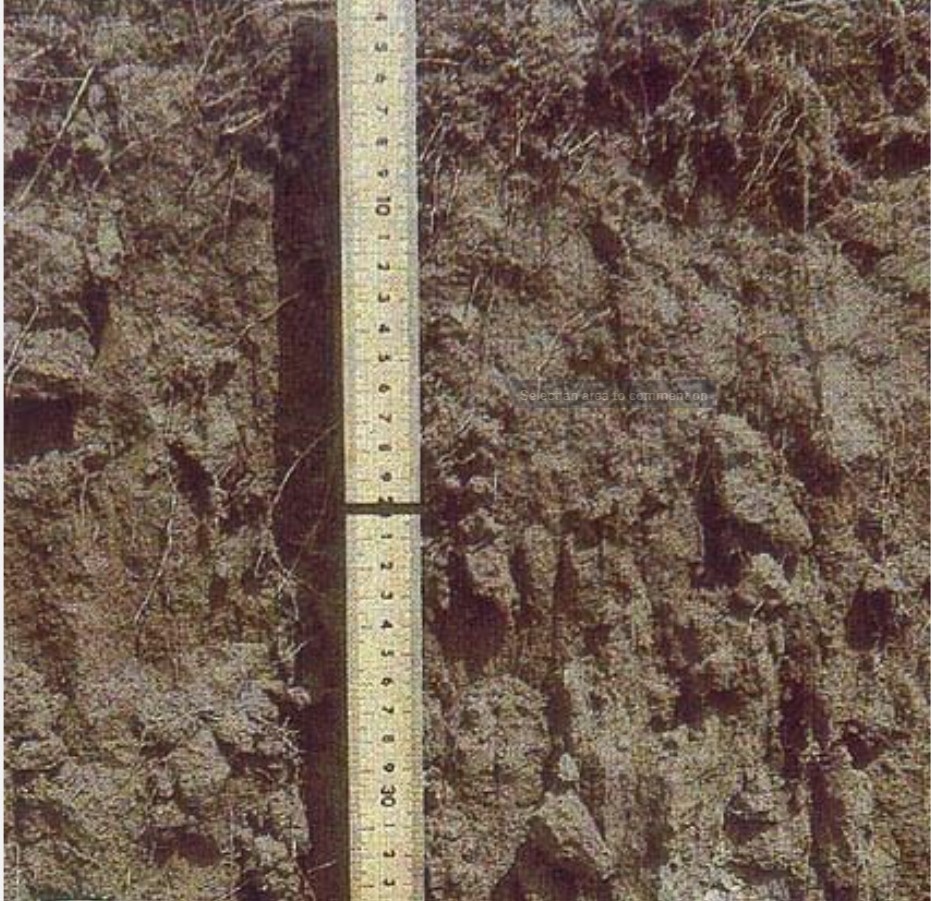
Soil Classification Working Group. 1998. The Canadian System of Soil Classification, 3rd ed. Agriculture and Agri-Food Canada Publication 1646. ISBN 0-660-17404-9
- Cryosolic soils Crysolic soils occupy areas where the permafrost lies close to the surface. They are predominant in the tundra but are also common as fine-grained soils in subarctic forests extending into the boreal; they also occur in some alpine regions. Permafrost is not a completely frozen solid block of ice; however, lenses and pods of ice in the soil influence the movement of water through the soil. Because water migrates from areas of higher potential to areas of lower potential, the ice can cause water to move in directions that are contrary to the influence of gravity, resulting in mixed or broken horizons (Figure 6.8).
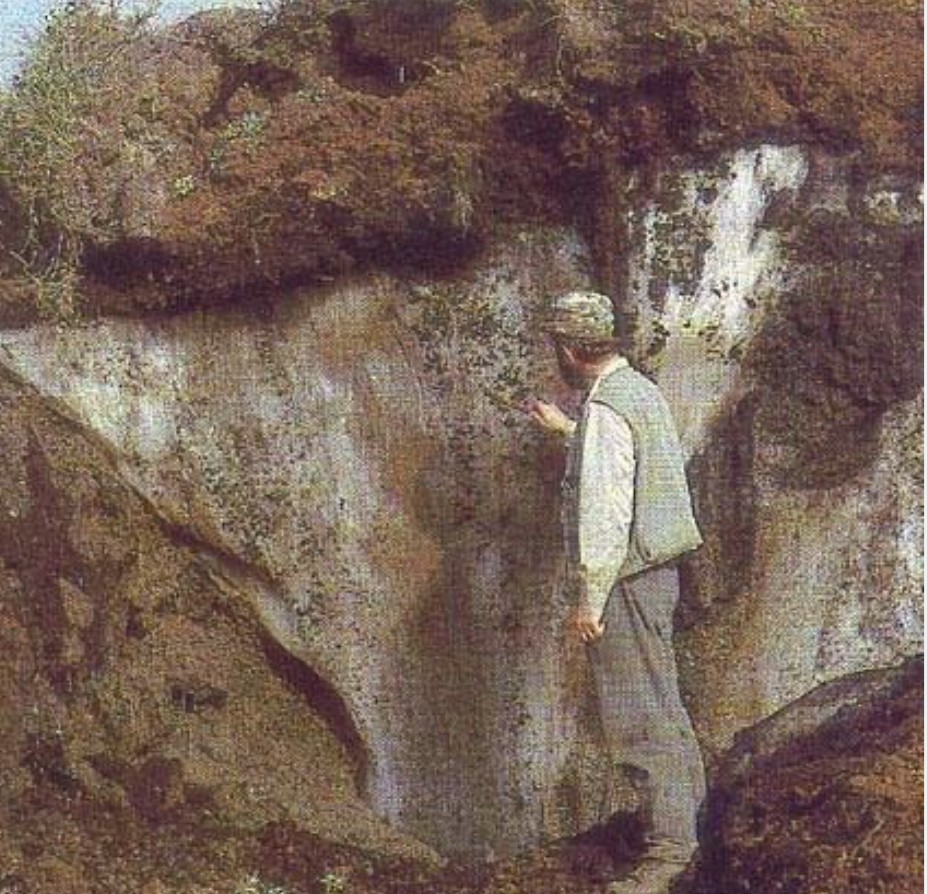
Soil Classification Working Group. 1998. The Canadian System of Soil Classification, 3rd ed. Agriculture and Agri-Food Canada Publication 1646. ISBN 0-660-17404-9
- Gleysolic soils Gleysolic soils experience episodic or prolonged periods of water saturation, creating a reducing environment due to lack of aeration (Figure 6.9). Gleyed A, B, or C horizons range in colour from dull grey to bluish or greenish grey, indicating prolonged saturation. Localized oxidation and reduction of hydrated iron oxides produce rust-coloured mottles, also indicating prolonged saturation. Although usually occurring in saturated depressions, gleysolic soils are rarely the dominant soil type in an area (Figure 6.9).
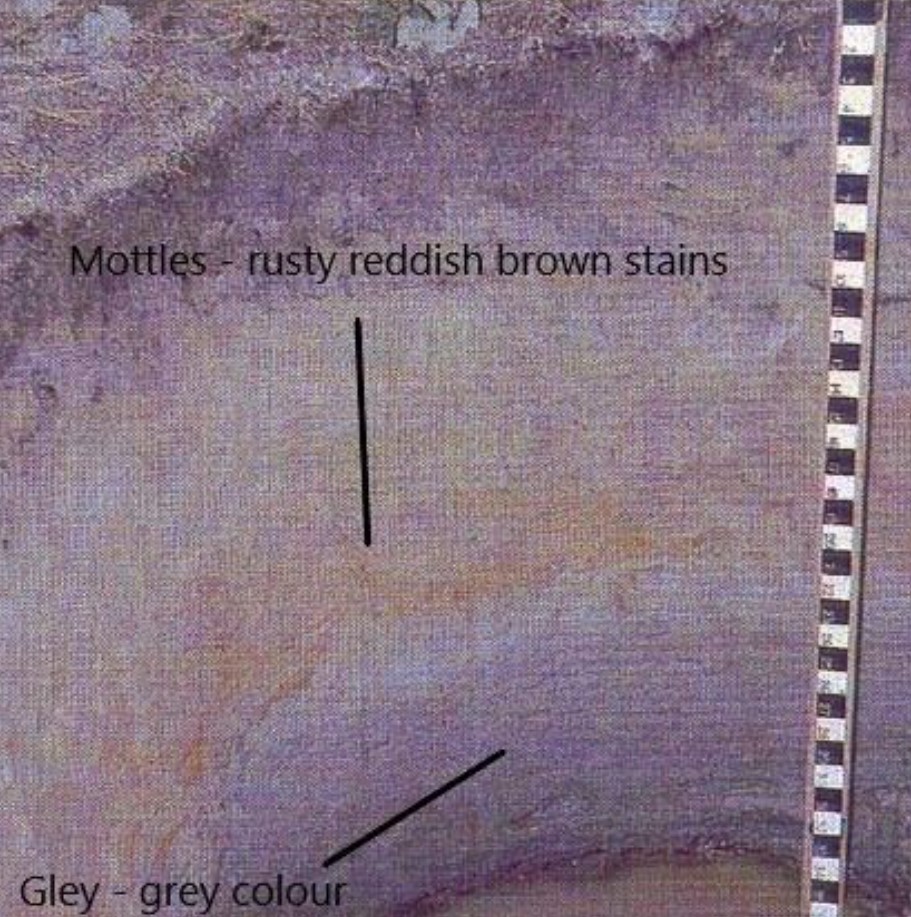
Soil Classification Working Group. 1998. The Canadian System of Soil Classification, 3rd ed. Agriculture and Agri-Food Canada Publication 1646. ISBN 0-660-17404-9
- Luvisolic soils Luvisolic soils develop in poorly to well-drained sites under forests in a range of climates. They generally have a light-coloured A horizon and darker B horizons with clays dominant (Figure 6.10).
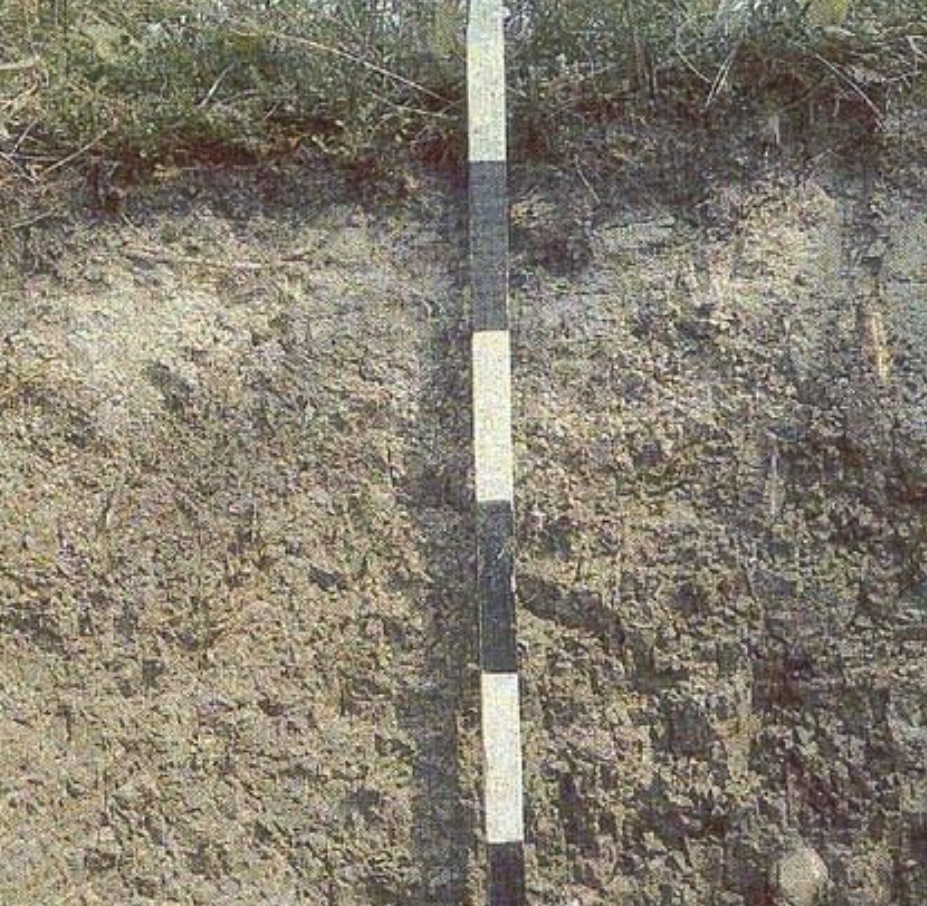
Soil Classification Working Group. 1998. The Canadian System of Soil Classification, 3rd ed. Agriculture and Agri-Food Canada Publication 1646. ISBN 0-660-17404-9
- Organic Soils Organic soils consist of 30% or more organic matter and are saturated for prolonged periods in marshes, bogs, or swamps (Figure 6.11).
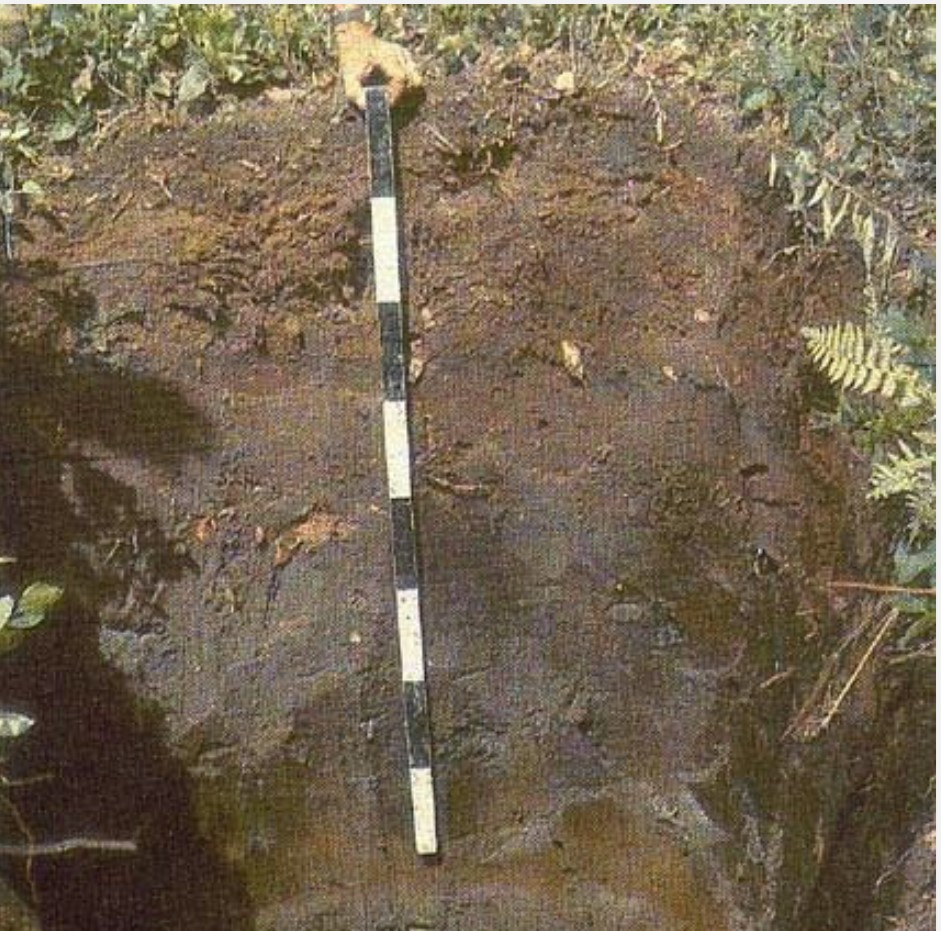
Soil Classification Working Group. 1998. The Canadian System of Soil Classification, 3rd ed. Agriculture and Agri-Food Canada Publication 1646. ISBN 0-660-17404-9
- Podzolic soils Podzolic soils develop in poorly to well-drained sites under forest cover in cool or very cold climates. They usually have a grey layer near the top with a reddish brown to black B horizon containing significant humus (organic material) along with iron and aluminum oxide precipitated from leach water (Figure 6.12). They are extensive on the Canadian Shield.
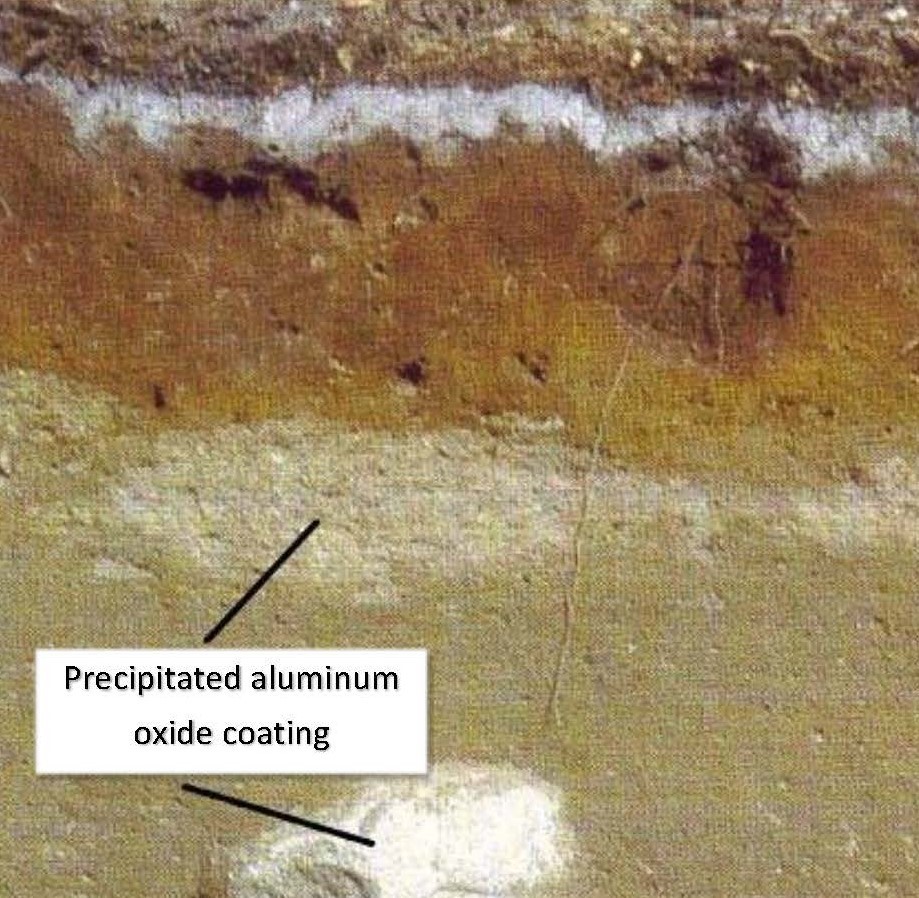
Soil Classification Working Group. 1998. The Canadian System of Soil Classification, 3rd ed. Agriculture and Agri-Food Canada Publication 1646. ISBN 0-660-17404-9
- Regosolic soils Regosolic soils develop in poorly to well-drained sites. Their soil profiles are not well developed, with only slightly altered (weathered) parent rock. The B horizon is usually thin or absent but may have an organic layer at the surface or a poorly developed humus-rich A horizon (Figure 6.13). They are most prominent under tundra vegetation.
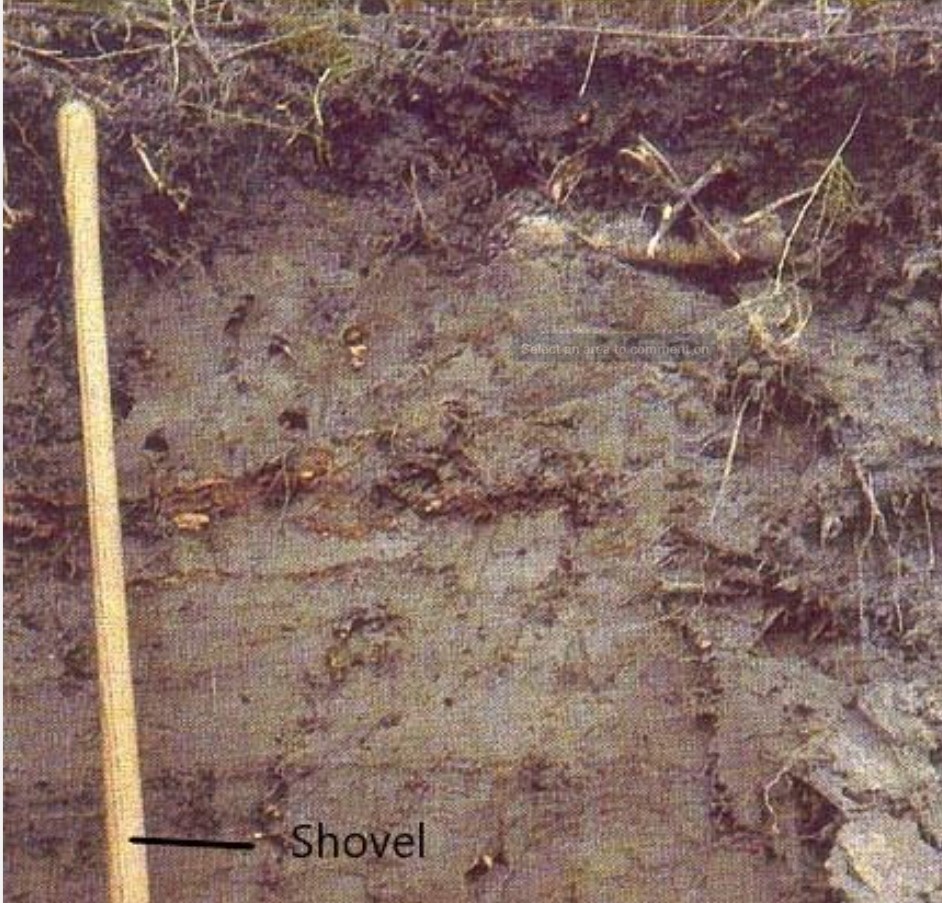
Soil Classification Working Group. 1998. The Canadian System of Soil Classification, 3rd ed. Agriculture and Agri-Food Canada Publication 1646. ISBN 0-660-17404-9
- Solonetzic soils Solonetzic soils occur in poorly to well-drained areas. Their physical and chemical features result from salination (the parent material may be saline — that is, salty — or the soils themselves may be saturated with saline water) followed by desalination and leaching. Organic matter and clays accumulate in the B horizon, and continued leaching can produce salt accumulation in the C horizon (Figure 6.14). Solonetzic soils are found mainly in the grassland areas of the plains.
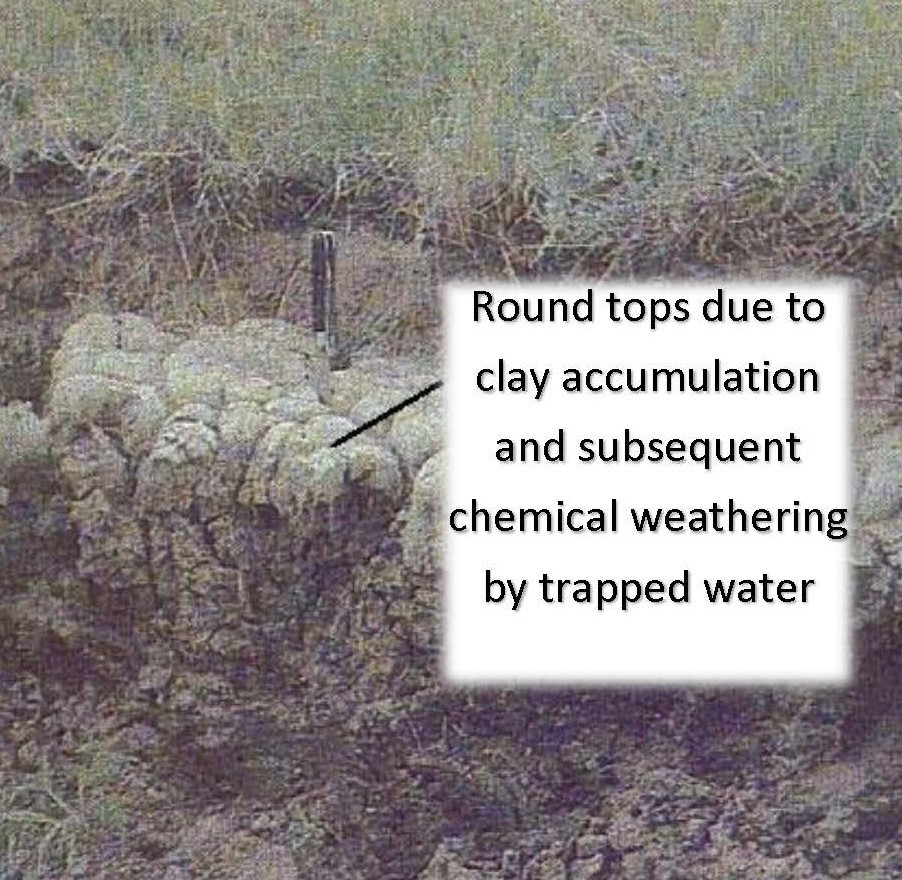
Soil Classification Working Group. 1998. The Canadian System of Soil Classification, 3rd ed. Agriculture and Agri-Food Canada Publication 1646. ISBN 0-660-17404-9
- Vertisolic soils Vertisolic soils are clay-rich soils that have a high shrink potential when dry and high swell potential when wet. This creates shear planes that prevent the formation of horizons because leaching is inhibited (Figure 6.15). They are usually light-coloured and form in arid regions of the plains.
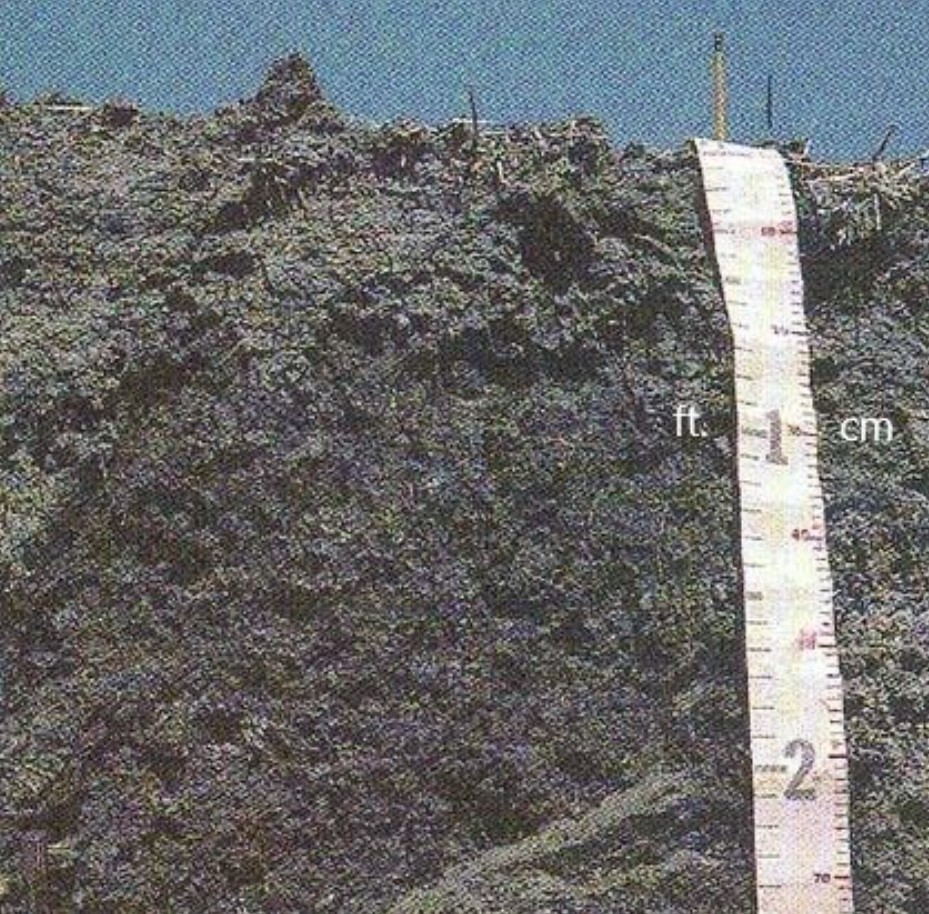
Soil Classification Working Group. 1998. The Canadian System of Soil Classification, 3rd ed. Agriculture and Agri-Food Canada Publication 1646. ISBN 0-660-17404-9
6.3 Mass Wasting
As seen previously, the process of weathering leaves behind loose heterogenous materials of soil and fragmented rock that are susceptible to mass movement under the influence of gravity. Therefore, all processes that cause the movement of soil and rock downslope are called mass wasting. When soil and fragmented rock exist on a slope, they will not move until the downward force exceeds the forces (such as friction and cohesion) holding them in place.
Factors Controlling Mass Wasting
The nature of the slope materials, the steepness of the slope, and the amount of water present are the main factors controlling mass movement of soil and rock downslope.
Slope Materials
Consolidated material will generally be more resistant to movement than unconsolidated material. Consolidated material consists of massive or jointed rock, while unconsolidated materials are either soils or mixtures of soil and fragmented rock. The main parameter here is the size of the individual solid pieces (grains and particles). Unconsolidated material will be stable on a particular slope depending on the angle of internal friction (also known as the angle of repose). This is the natural angle at which the particles will come to rest. Imagine you have a bucket of sand and you pour it onto a flat table, creating a conical mound. Looking at the mound from the side, the angle of the slope is the angle of repose. The steepness of this angle depends on the friction between the individual grains, which is why it is also known as the angle of internal friction. The larger the grains, the more surface area available for friction and therefore the steeper the angle. Also, more angular grains of the same size will produce more friction (due to more surface area) and therefore create steeper angles than will more rounded grains. (See Video 6.1.)
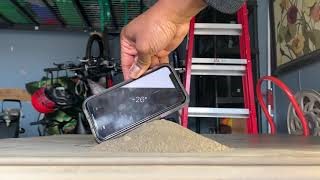
Click on the image to load the video.
Steepness of the Slope
When soil and rock exist on slopes whose angle exceeds their angle of internal friction, they tend to fail and move downslope to achieve stability (see Video 6.2 and Figure 6.16). That is, they constantly search for the stability of the natural angle of repose (angle of internal friction). Conversely, if soil and rock exist on a slope whose angle is smaller than their internal angle of friction, then they are stable and will never move unless their nature is changed by the presence of water. Therefore, the steepness of the slope on which soils and rock occur will greatly influence mass movements.
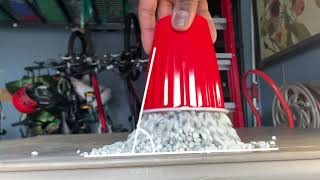
Click on the image to load the video.
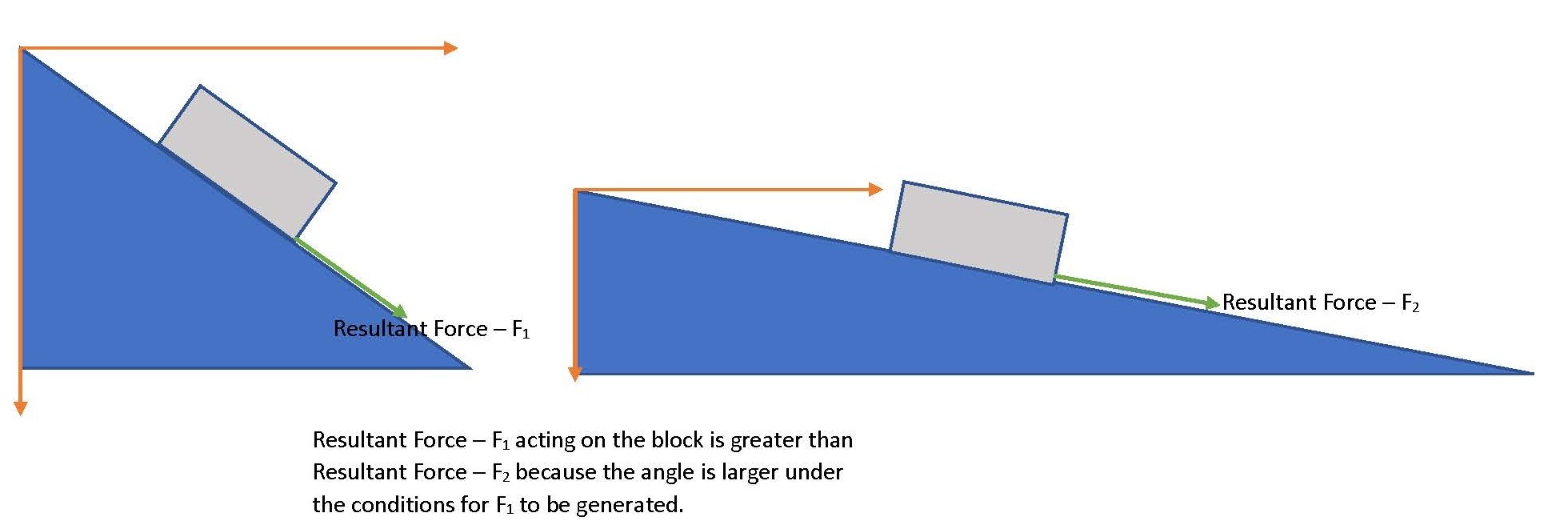
Resultant force F1 acting on the block is greater than resultant force F2 because the angle under the conditions to generate F1 is larger
Amount of Water
The water content of soil and rock can affect their mass movement downslope by reducing the effect of friction. Think back to the last time you walked through the halls of your college and recall the “Slippery When Wet” signs posted on a freshly mopped floor. Water is an excellent lubricant that reduces the friction between your feet and the floor, increasing the chances of slipping. So too does water reduce the effect of friction between the individual grains in soil and rock, reducing the internal angle of friction. This then reduces the steepness of the angle at which the material will be stable. For example, saturated sand will be stable at an angle far smaller than will dry sand (Video 6.3).
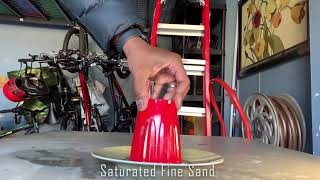
Click on the image to load the video.
Partially saturated slope materials will have a counterintuitive property of cohesion that resists movement. Their resistance to motion is a result of the apparent cohesion between adjacent grains in partially saturated soils. Partial saturation creates a thin film of water around each grain, and these films connect when the grains are in close proximity. The result, because of surface tension, is one congruous film that acts like an elastic band binding the grains together (Figure 6.17). Therefore, the natural angle of repose is significantly increased by the “stickiness” of the grains.
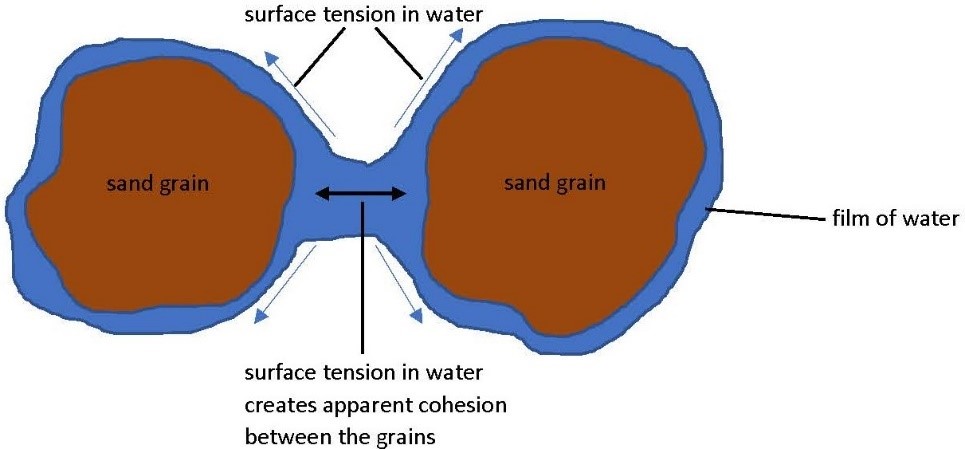
Surface tension in water arises from the abundance of bonding between the polarized water molecules (H+ OH–) at the water–air interface (the surface). Water molecules in the water column would have equal amount of bonds in all directions while those at the surface will have extra bonds to the sides (Figure 6.18).
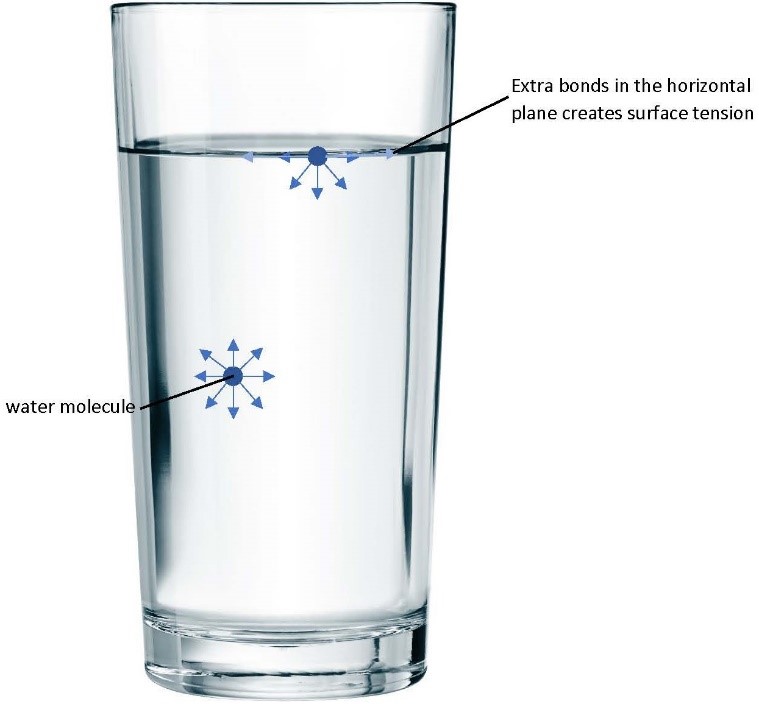
Because force (weight) = mass × the acceleration due to gravity (which is a constant), the addition of water also increases the mass of the soil and therefore has the added effect of increasing the weight (force acting on the slope material) of the soil and rock, increasing the potential for mass movement (see Figure 6.16 above).
6.4 Classification of Mass Movements
Mass movements are classified based on the nature of the slope materials, the nature of the downslope movement, and the speed of the movement (Table 6.2).
Nature of the Slope Materials Whether slope materials are consolidated or unconsolidated is a major factor in the type of mass movement that can occur. Consolidated slope materials are made of rock that is massive (that is, it has no cracks or joints) or those that are jointed, fractured, or layered (bedded). Because consolidated materials resist movement, their failure and subsequent movement downslope is usually catastrophic, which means it moves at moderate to high speeds with high energy (turbulence). Unconsolidated slope material is made of soil and/or rock fragments that have a higher potential to move under the influence of gravity. Therefore, their downslope movements can range from very slow with low energy to high speeds with high energy.
Nature of the Movement This is a physical description of the way in which slope materials move downslope and encompasses flows, sliding, and falling. Flow describes the movement of the material like a fluid; slide describes movement along a shear plane parallel to the slope; and falling describes movement through the air from higher potential energy to lower potential energy.
Speed of the Movement Slope materials can move from very slowly, at speeds of the order of centimetres per year, to very fast, at several kilometres per hour. Speed constrains the type of movement possible, depending on the nature of the slope material. At slow speeds (1 cm/year), unconsolidated material will creep, while at moderate speeds (1 km/hr), unconsolidated material will flow or slide. Flows are initiated by high rainfall causing the soil to behave more like a fluid than a solid. Furthermore, unconsolidated material will avalanche at high speeds (in excess of 5 km/hr). An avalanche occurs when there is a significant amount of air in the flow that holds the particles in suspension and lubricates the flow. This causes avalanches to move at high speeds. Consolidated material does not move at slow speeds and will slide only at moderate speeds. At high speeds, consolidated material will either fall or avalanche.
Table 6.2. Classification of Mass Movements. Click on each classification to see an example.
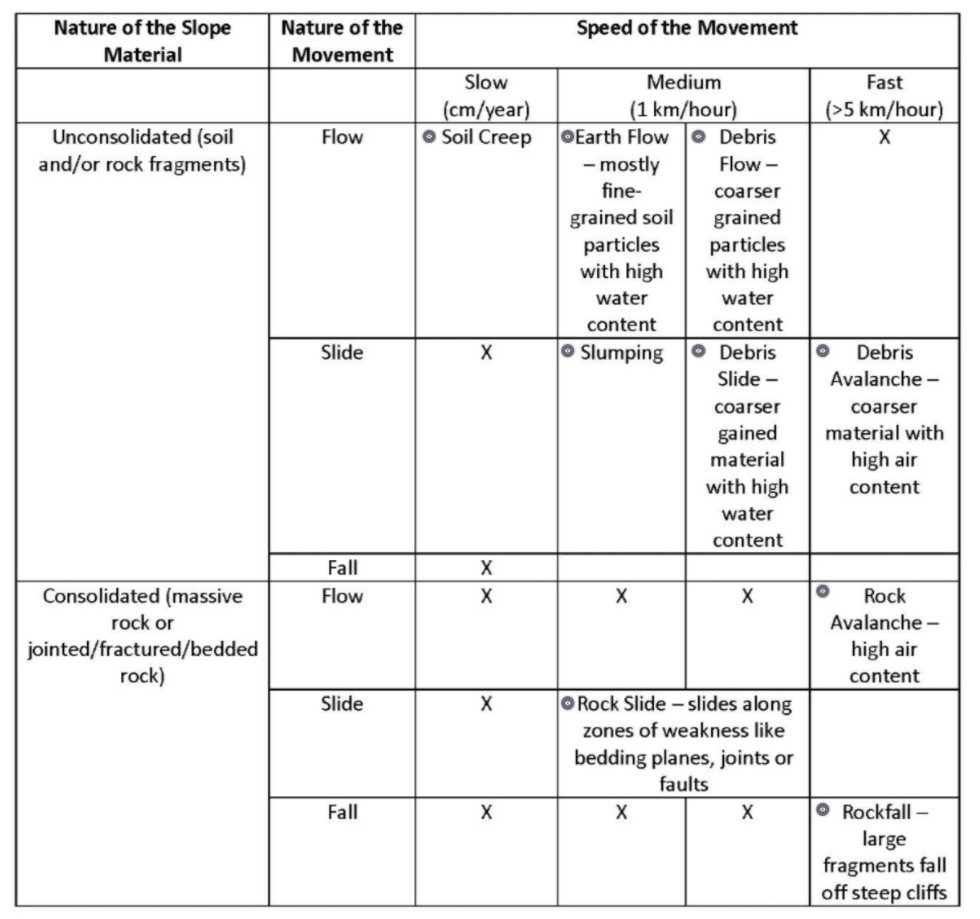
6.5 The Niagara Escarpment: A Case Study in Weathering
An Overview of Ontario Geology
The first North American continent, Arctica, formed about 2.5 billion years ago. This continent that included Ontario coalesced out of the binding together of older geologic provinces, which is a term introduced in the early 1860s by pioneer Canadian geologist Sir William Logan (1798–1875). These provinces — the Slave, Hearne, Superior, Rae, and Wyoming — formed during the Archean Eon. Over the last 2.5 billion years, Ontario has been a part of at least four major continents: Arctica, Nena (today’s northern Europe and North America), Rodinia, and Pangaea.
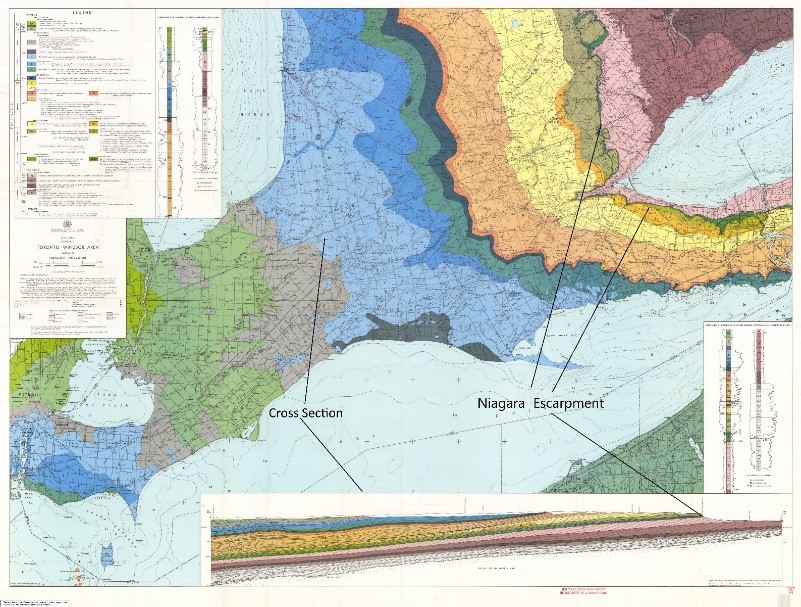
The Superior Province of northern Ontario was brought together by either one or a sequence of orogenies about 2.7 billion years ago. An orogeny is an episode of rock deformation due to compressional forces associated with convergence, and results in the development of large mountains. These mountains will have been long since eroded, leaving behind their “roots” as the rocks that underlie most of the northern parts of Ontario. These rocks are mineral rich and account for a significant amount of Canada’s mineral and metal production.
The other provinces that make up the geologic provinces of Ontario are the Southern and Grenville Provinces. The Superior, Southern, and Grenville Provinces make up the Canadian Shield (Sequence 1; see Figure 6.20), which contains the oldest rocks in Ontario and forms the platform upon which the subsequent younger rocks are deposited.
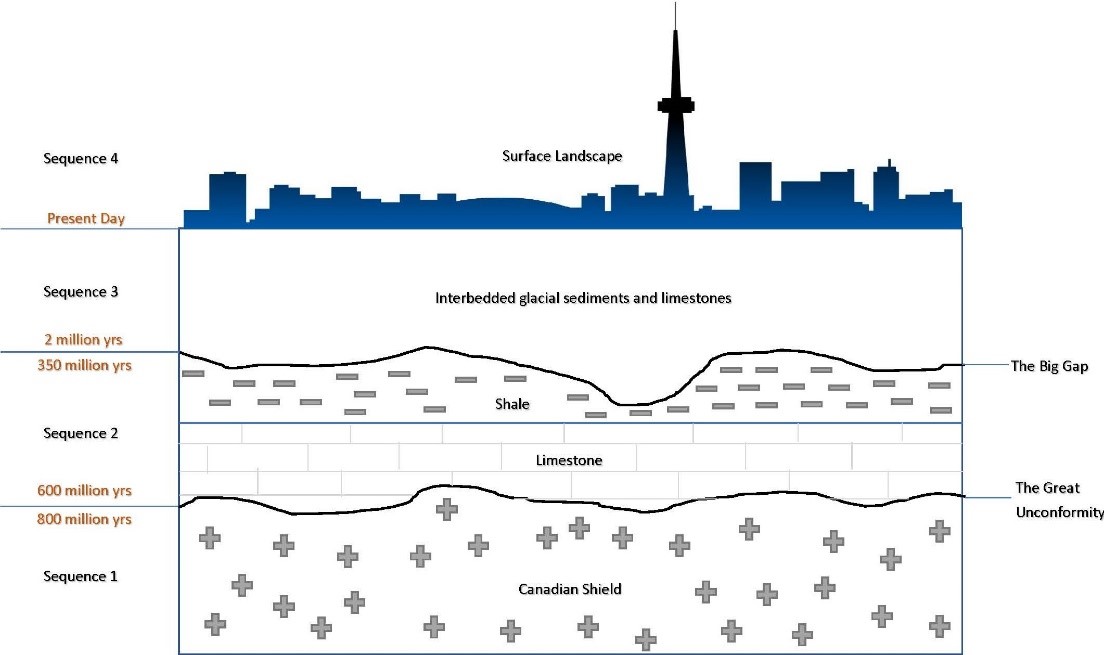
Between about 800 million and 600 million years ago, there was an erosive sequence followed by no deposition, resulting in the development of the Great Unconformity (Video 6.1). This was followed by the deposition of Paleozoic sedimentary rocks (limestones) laid down between 600 million and 350 million years ago in ancient warm seas (Sequence 2; see Figure 6.20). These shallow marine seas inundated a bowl-shaped depression centred on the Upper Michigan peninsula, creating rocks from sediments that had been laid down in this shape. The differential weathering rates of these rocks are responsible for the development of the Niagara Escarpment. The rocks contain fossils of trilobites, gastropods, crinoids, and brachiopods and are thus said to be fossil rich, or fossiliferous (see Video 6.4).
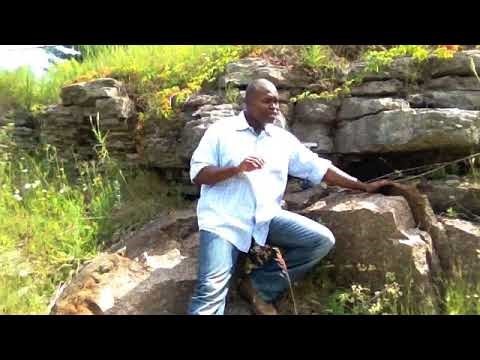
Click on the image to load the video.
Between 350 million and 2 million years ago, another erosive, non-depositional sequence occurred, producing an unconformity known as the Big Gap. This was then followed by the deposition of Pleistocene glacial sediments (Sequence 3; see Figure 6.20) laid down by continental ice sheets during the several ensuing ice ages. Intermittent fossiliferous rocks were deposited between ice ages and are therefore interbedded with the glacial sediments. The youngest layer is the natural landscape, where we live, with a geomorphology that has been shaped by the last glaciation (Sequence 4; see Figure 6.20).
The Niagara Escarpment
The Niagara Escarpment is not confined to the Niagara region but runs from New York State all the way through Grimsby, up the Bruce Peninsula, through Manitoulin Island and down to Wisconsin and Illinois (Figure 6.21). It gets its name from the Niagara River because it includes the cliff over which the river plunges, forming the Niagara Falls (Video 6.5).
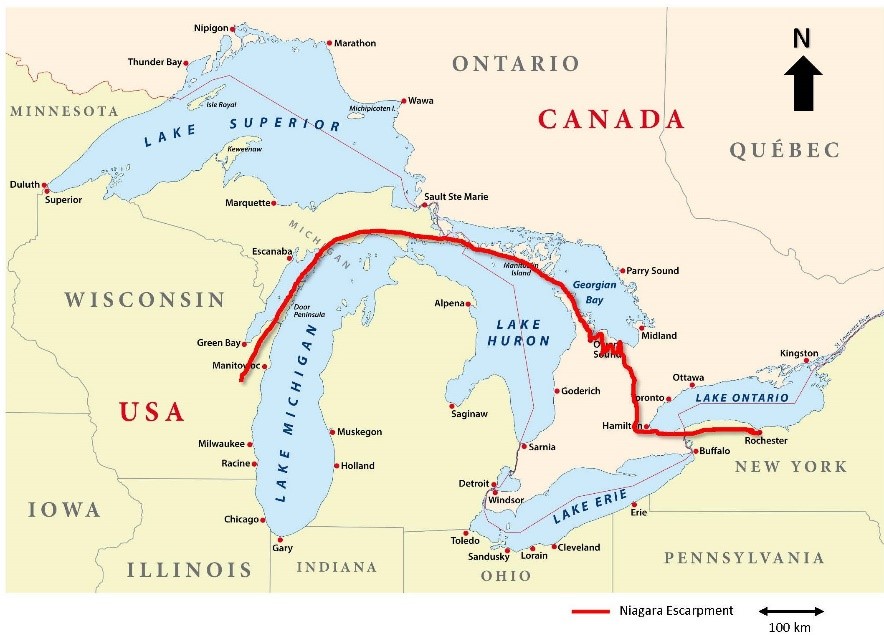
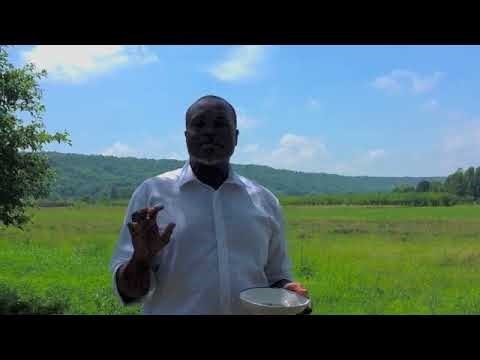
Click on the image to load the video
The Niagara Escarpment was formed by differential erosion rates of the rocks in Sequence 2 of the generalized cross-section. These are the sedimentary rocks that are exposed in this region (Figure 6.22). Specifically, the cap rock of the formation is harder and more resistant to weathering and erosion than the underlying shale. The cap rock is a dolostone made of dolomite, which is a limestone with the calcium replaced by magnesium. This stratigraphic sequence is exposed to erosion due to its inclined profile. The inclined profile is due to the edges of the ancient Michigan basin being exposed at the surface. The Michigan basin is bowl shaped depression, in the Earth’s crust that is centred on the present-day Michigan Peninsula (see section 5.4).
Although the rocks that make up the Niagara Escarpment are approximately 430 million years old they form just part of the rim of the Michigan basin with rocks as old as 500 million years. After the Great Unconformity, the Michigan basin was formed ~ 600 million years ago when sediments were laid down at the bottom of a warm shallow sea that inundated a depression in the Earth’s crust, centred on the Michigan peninsula. As the rock layers built up, filling the depression, another erosive sequence followed by a period of non-deposition occurred creating the Big Gap. Therefore, only rocks as young as 350 million years old survive.
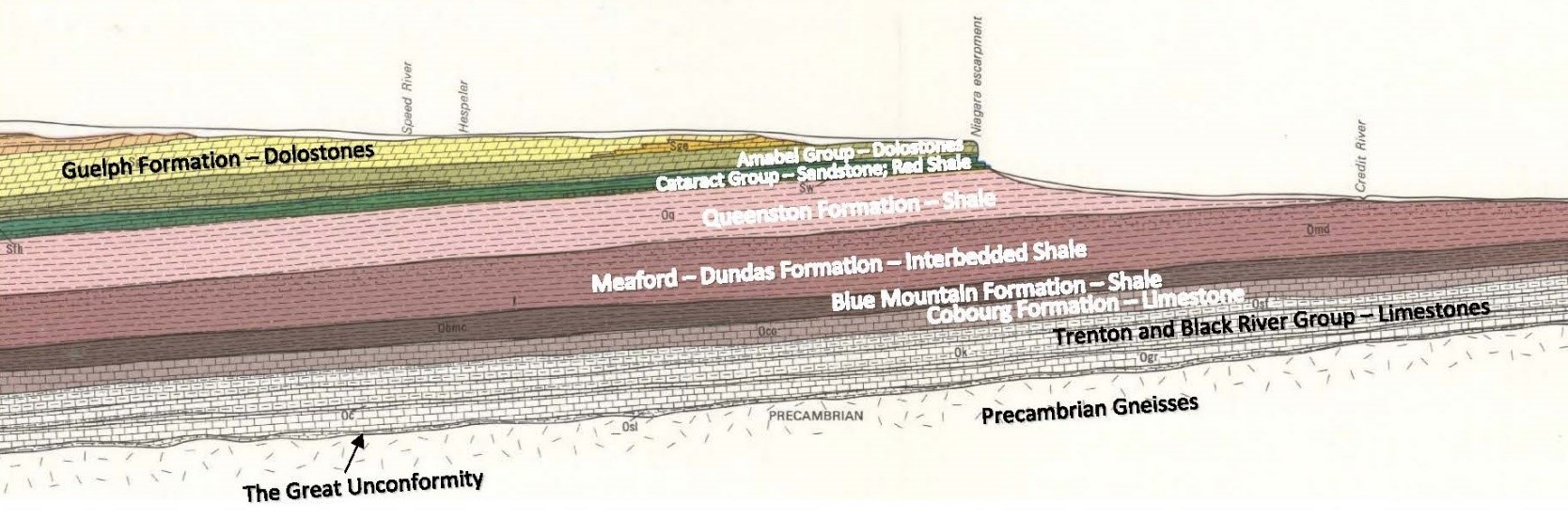
The process that breaks down rock at the surface of Earth
Chemical reactions that break down rock when minerals come in contact with air and water
The reaction of minerals with oxygen from air or water, altering the mineral structure
A chemical reaction of a substance with water
Describing conditions in which insufficient rainfall leads to extremely dry conditions
The process by which water entering zones of weakness in rock freezes, opening up a larger crack
The exposure of rock by the removal of other strips from its surface
For soils, processes changing materials from one form to another
For soils, processes causing the movement of materials from one zone to another within the soil
A measurement of the hollow spaces in between grains that are occupied by water, air, or a combination of both
The zones of a soil profile, defined by their contents
The top zone of a soil profile, consisting of the humus layer, quartz, and clays
An organic rich soil layer
The middle zone of a soil profile where soluble minerals are oxidized and deposited
The hollow spaces in between grains that are occupied by water, air, or a combination of both
The zone in a soil profile dominated by bedrock altered by weathering
Is the zone in a soil profile dominated by unaltered/unweathered bedrock
Soils that evolve in one place
Soils that are eroded from slopes and carried downhill
Categories of soil taxonomy
Residual soils with a brownish B horizon
Soils with high carbonate concentration and a dark A horizon
Permafrost soils
Soils with greyish or mottled A, B, or C horizons due to prolonged or intermittent lack of aeration
Clay-dominant soils with a light A horizon and dark B horizons
Soils of 30% or more organic matter
Soils with a grey layer near the top and with humus containing iron and aluminum oxide
Soils with poorly developed profiles featuring slightly altered parent rock
Soils with significant salt accumulations
Clay-rich soils with high shrink potential when dry and high swell potential when wet
Processes that cause the movement of soil and rock downslope
A property of sticking together due to electrostatic forces
The fluid movement of matter
Movement of matter along a shear plane parallel to a slope
The very slow movement of unconsolidated material down a slope
(n.) The rapid fall of material down a mountainside or cliff face; (v.) to tumble as an avalanche
An episode of mountain building due to compressional forces associated with convergence
The basement rocks of the Precambrian age in Eastern Canada and the northeastern United States characterized by granitic igneous rocks and gneisses of igneous origin
The result of a period of erosion then non-deposition ~800 million to ~600 million years ago
An unconformity created by a period of erosion followed by non-deposition 350 million to 2 million years ago